Pharmaceutical Residue-Derived Modified Fuels: Thermochemical Conversion Laws and Mechanisms
In recent years, the pharmaceutical industry in our country has developed rapidly and has become an important part of the national economy. The nearly 3-year-long COVID-19 pandemic has further stimulated the rapid growth of this industry. According to statistical data, the total revenue of China's pharmaceutical industry in 2022 exceeded 33.6×10^8 CNY. Traditional Chinese medicine and antibiotics are the two main products of the pharmaceutical industry, derived from the extraction and refinement of effective medicinal components in the pharmaceutical process, which inevitably produces a large amount of residual residue, commonly known as drug residue, mainly divided into two categories: traditional Chinese medicine residue and antibiotic bacterial residue. Our country is the birthplace of traditional Chinese medicine and the largest producer/supplier of antibiotics. According to relevant literature reports, the annual accumulation of discarded traditional Chinese medicine residue and antibiotic bacterial residue reaches several million to tens of millions of tons. With the increasing attention and urgent demand for medical health, the rapid annual increase in the amount of drug residue in our country is an inevitable trend. The effective and safe disposal of these residues has become a pressing issue for enterprises and a hot topic of general concern in the industry. Drug residue has both "waste" and "resource" dual attributes: on one hand, it belongs to typical light industry organic solid waste, with a high initial moisture content (mass fraction over 70%), and contains certain pathogens, mycotoxins, residual antibiotics, and metabolic products, which can easily deteriorate and rot, making it impossible to use directly. Without proper disposal or using traditional methods such as regional stacking, on-site landfill, and open-air burning, it will cause varying degrees of pollution to soil, water bodies, and air, and may even endanger human health. On the other hand, drug residue also falls under the broad category of biomass, originating from specific light industrial production processes. The dry state of drug residue is rich in hemicellulose, lignin, proteins, and polysaccharides, with a high calorific value, making it a concentrated high-quality biomass resource, which has the potential to be used as a renewable raw material for the preparation of bio-based products. Therefore, from the perspective of environmental protection and resource conservation, achieving clean and efficient utilization of drug residue is very necessary and plays a positive role in our country's strategic deployment of "strengthening solid waste disposal, promoting the recycling of resources, and advancing ecological civilization construction."
Pharmaceutical residue, after thermochemical conversion, is used to prepare solid, liquid, and gas-derived fuels, which are applied in the thermal/power industry. This is an effective and direct way of resource utilization based on the current level of technological development and is also a hot topic in scientific research and engineering applications. This article focuses on two types of pharmaceutical residues, with the aim of energy utilization through thermochemical conversion, targeting solid, liquid, and gas fuels as end products. It reviews the current research progress related to preparation strategies, process technologies, and characteristic patterns of pharmaceutical residue-derived fuels. By comparing and analyzing the advantages and disadvantages of various energy application methods for pharmaceutical residues, it provides reference and feasible ideas for the research and application of clean and efficient use of pharmaceutical residues.
01
basic physicochemical properties of medicinal residue
As shown in Figure 1, traditional Chinese medicine residue is the organic solid remainder after a series of processes such as screening, steaming, and water or alcohol extraction to obtain effective components from medicinal plants. It mainly includes primary herbal residues (roots, stems, leaves, flowers, fruits), secondary animal-derived nutrients, and medicinal components. Based on its source, it can be known that the main biochemical components of traditional Chinese medicine residue are lignin (with a mass fraction exceeding 80%), cellulose, and hemicellulose, rich in organic matter, with typical characteristics of lignocellulosic biomass. Antibiotic mycelial residue is a solid waste produced during the production process of fermentation-based drug raw materials, mainly composed of mycelium, residual culture medium, biological metabolites, and a small amount of residual antibiotics. Its main components are proteins, amino acids, lipids, and carbohydrates, falling within the category of non-lignocellulosic biomass.
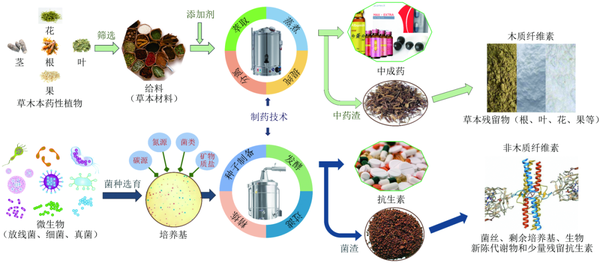
Figure 1
pharmaceutical process and procedure
From a biochemical composition perspective, compared to antibiotic mycelial residue, Chinese herbal medicine residue has a more stable organic functional group structure. Figure 2 summarizes the basic fuel characteristics of the two types of medicinal residues.
As shown in Figure 2, in the dry basis state, the two types of medicinal residue have high organic matter content, with a calorific value reaching 15~20 MJ/kg, and the fixed carbon (mass fraction 13%~16%) and volatile matter (mass fraction 76%~79%) are basically similar. In addition, the mass fractions of carbon and hydrogen elements are not much different, being 42%~43% and 5%~6%, respectively. It can be seen that although the two types of medicinal residue have different biochemical compositions due to their sources, from the perspective of elemental and industrial composition, the differences are small, possessing good resource attributes, and have prospects in high-value biobased fuels and carbon-based products.
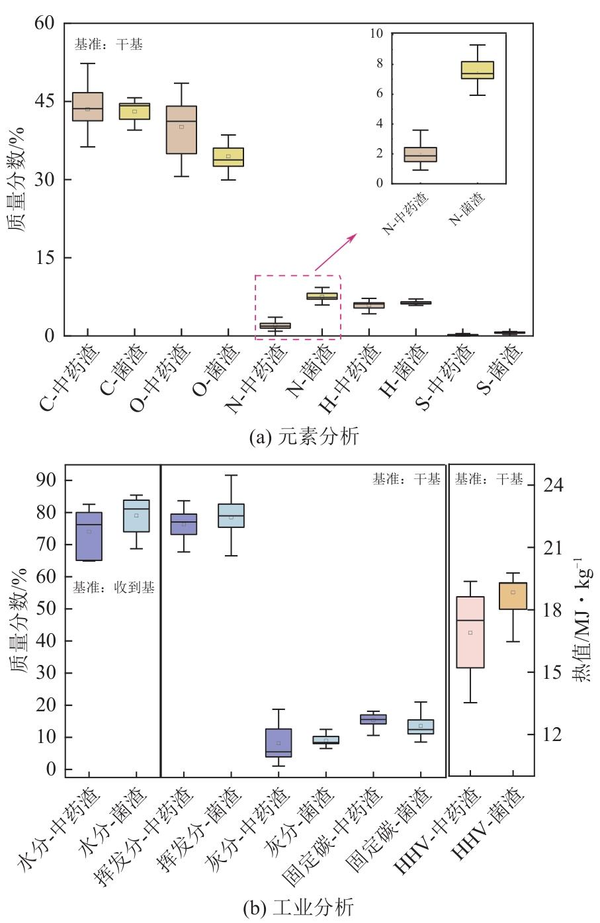
Figure 2
medicinal residue fuel characteristics
At the same time, it can also be seen from Figure 2 that the medicinal residue contains some harmful or useless components that are not conducive to energy conversion, such as moisture, ash, nitrogen, oxygen, and sulfur. The as-received moisture content of the two types of medicinal residues is very high, which is determined by the wet environment of the pharmaceutical process, while the ash content in the dry basis state is at a lower level; the oxygen content of the two types of medicinal residues is abundant, and the sulfur content is extremely low, similar to conventional biomass; it is particularly noteworthy that both types of medicinal residues contain much higher nitrogen content than general herbaceous biomass, especially antibiotic mycelial residues, with a mass fraction reaching 7%~9%, mainly due to the dual effects of biological nitrogen fixation during the growth process of medicinal plants and external nitrogen addition during the microbial fermentation process. These components reflect the waste attributes of the medicinal residues, and the related element migration, transformation, and product quality issues in the thermochemical conversion process are key constraints for the clean and efficient utilization of medicinal residues for energy. In addition, the residual components in medicinal residues can have a significant impact on the environment. Meng et al. chose the medicinal residue from digestive tablets to conduct an antibacterial experiment on Lactobacillus plantarum and found that its fermentation supernatant still has strong anti-Helicobacter pylori activity; Hong et al. used response surface methodology to investigate the hydrothermal liquefaction of penicillin fermentation residue for bio-oil preparation, and the residual microorganisms still had strong activity, with direct landfill disposal disrupting the local microbial community balance; Yuan et al. explored the binding characteristics of dissolved organic matter and Cu in medicinal residues, and the results showed that the resulting protein-like substances could deteriorate water quality and pollute the environment. Pesticide and solvent residues in traditional Chinese medicine residues, as well as residual antibiotics and antibiotic resistance genes in antibiotic mycelial residues, are also important considerations for the clean energy utilization of medicinal residues.
02
Characteristics and enhancement strategies of solid fuel derived from medicinal residue
Medicine residue, as a typical type of biomass waste derived from light industrial production, can be used as a solid fuel for thermal utilization. However, some inherent defect properties of medicine residue, such as high moisture content, high nitrogen, and high oxygen, are the key factors restricting its direct energy utilization. Currently, research on solid fuels derived from medicine residue mainly focuses on three aspects: direct combustion or co-combustion with other solid fuels, dehydration and quality improvement of medicine residue, and torrefaction modification of medicine residue.
2.1
Characteristics and enhancement methods of direct combustion of medicinal residue
Regarding the existing research on direct combustion of medicinal residue, the focus is mainly on antibiotic bacterial residue, with combustion behavior and emission characteristics being the key points of study. Yang et al. used thermogravimetric-Fourier transform infrared spectroscopy and pyrolysis-gas chromatography/mass spectrometry to investigate the combustion patterns of penicillin bacterial residue, finding that the combustion process can be divided into three stages: sequential thermal decomposition of components, thermal oxidative cracking, and mineral migration, with the release of major carbon- and nitrogen-containing volatiles occurring in the first two stages. Zhang et al. used a small-scale fluidized bed reactor to study the pollutant emission characteristics of a specific type of bacterial residue (high ash, rich in nitrogen, and sulfur) during combustion, discovering that gaseous pollutants (NO, SO2) can be effectively suppressed through the catalytic action of water vapor and ash components, though their emissions still exceed standards. To address the issues of excessive pollutant emissions and poor combustion efficiency mentioned above, some novel combustion technologies have been practiced by predecessors, such as decoupled combustion and co-combustion. Chen et al. pointed out that under conditions of higher temperatures (>873K) and lower O2/fuel ratios (<11L/g), decoupled combustion, based on the redox mechanism of pyrolysis gas and char, can significantly reduce NO emissions from nitrogen-rich bacterial residues, and compared to traditional direct combustion, it exhibits superior thermal stability. In addition, some studies have reported that a certain amount of bacterial residue co-combusted with coal undergoes synergistic reactions, reducing the activation energy required for combustion, allowing the complete combustion of heavy volatiles and fixed carbon in low-rank coal, thus increasing its calorific value. Through kinetic analysis of the combustion processes of both, it was found that the combustion process can be divided into low-temperature and high-temperature stages, possibly due to the large heat release from the combustion of volatiles contained in bacterial residues at low temperatures, which increases the heating rate of coal, promoting more thorough coal combustion. Wang et al. further studied the co-combustion system of bacterial residue and coal slurry, indicating that bacterial residue and coal particles can be connected via hydrogen bonds of polar functional groups, increasing the viscosity of the coal slurry, preventing coal agglomeration, and lowering the ignition temperature of coal. Hong Chen et al. also reached similar conclusions through research on the co-combustion characteristics of antibiotic bacterial residue and coal, showing that the addition of bacterial residue can decrease the burnout index of pulverized coal combustion, enhancing the stability of the process. The co-combustion of plant-based biomass with bacterial residue has a similar effect; Wang et al. found that due to the high volatile content, bacterial residue has a low ignition point and high combustion temperature, and adding bacterial residue can accelerate the heating rate of the co-combustion system, shorten the combustion process, and improve combustion performance. Although co-combustion technology shows advantages in handling bacterial residue, most of the reported studies are limited to special types of bacterial residue with low moisture, nitrogen, and ash content. Concerning Chinese herbal medicine residue, Chen et al. used multiple models to study the reaction kinetics of Chinese herbal medicine residue under different CO2/O2 mixed atmospheres, pointing out that the reaction mechanism of Chinese herbal medicine residue combustion is not affected by the reaction atmosphere, and when the raw material conversion rate is below 30%, the energy transfer rate is faster; while when the conversion rate exceeds 80%, pores form in the reactant particles, facilitating the transfer of oxygen and energy to the interior of the particles. Additionally, this study analyzed the burned ash, finding that changing the combustion atmosphere only affects the relative content of elements in the ash, without significantly impacting the risk of slagging and fouling. Besides the studies focusing on the influence of the atmosphere on the combustion kinetics and ash characteristics of Chinese herbal medicine residue, there are few other reports on the direct combustion characteristics of Chinese herbal medicine residue. In general, previous studies on the direct combustion of medicinal residue have mainly focused on disposal based on the principles of reduction and harmlessness, especially for the effective utilization of antibiotic bacterial residue, with fewer studies on combustion enhancement methods, resulting in generally moderate effects in terms of improving efficiency and controlling pollution, making it not the primary choice for utilizing solid fuels derived from medicinal residue.
2.2
Characteristics and Mechanisms of Quality Improvement and Nitrogen Reduction through Thermochemical Pretreatment of Medicinal Residue
Medicinal residue has high initial moisture content, rich in nitrogen, and abundant in oxygen, which are defective characteristics. If used as an effective outlet for solid fuel, it is very necessary to use thermochemical pretreatment methods to improve its quality and reduce nitrogen. On one hand, dehydration pretreatment is one of the strategies to improve the quality of medicinal residue as a solid fuel. Most of the water molecules in medicinal residues (especially antibiotic mycelial residues) exist in the form of colloidal superparticles, making it difficult to remove using traditional mechanical methods (such as centrifugation and filtration). Currently, microwave radiation, hydrothermal treatment, and hydration salt dehydration have been reported as enhanced means to improve the dehydration performance of mycelial residues. According to literature, microwave radiation, due to its instantaneous and efficient heating, causes some cells in the microwave field to be locally heated, leading to a sudden increase in intracellular pressure in mycelial cells, exceeding the cell wall's tolerance limit, resulting in rupture and fragmentation. This cell-breaking effect can reduce the moisture content of the medicinal residue, but the effect is limited. Under optimal operating conditions (414W, 120s), the moisture content only decreases from 83.75% to 75.26%. Cai et al. further confirmed that although microwave radiation greatly affects the raw material particle size and intracellular polymer release related to dehydration performance, it cannot destroy the bound water in the matrix. Zhang et al. believe that hydrothermal treatment, due to its relatively mild conditions (below 200°C), can avoid the formation of tar on the surface of biofuels, which hinders the evaporation of internal moisture, and can convert the "bound water" inside the medicinal residue into free water, making it a more effective means for the dehydration of medicinal residues. Under optimized hydrothermal conditions (180~200°C, 30min), modified solid fuels with a moisture content below 50% (by mass) and a solid recovery rate of 38%~43% can be obtained; of course, if hydrothermal treatment is only aimed at dehydration, the harsh conditions of high temperature and high pressure will inevitably lead to high energy consumption. In recent years, some inorganic salts, such as Na2SO4, MgSO4, and Na2CO3, have attracted attention as energy-saving dehydrating agents for medicinal residues because they can spontaneously combine with the gel-like water contained in the medicinal residue to form hydrates when mixed at room temperature, thereby reducing dehydration energy consumption. On one hand, the dehydration mechanism conforms to the first-order kinetic equation and is mainly influenced by factors such as temperature, type of inorganic salt, and particle size. On the other hand, Na2SO4 crystalline hydrate has lower thermal stability and is easier to remove crystalline water upon heating. Using Na2SO4 can significantly reduce the temperature and energy consumption of the dehydration process and greatly shorten the reaction time. It is worth noting that although hydrated inorganic salts are a class of green and energy-saving dehydrating agents that can enhance the dehydration of medicinal residues, their recyclability and stability need to be considered when applied to actual medicinal residue disposal projects.
As another type of preprocessing method, baking technology can upgrade medicinal residue into a modified solid fuel more suitable for thermal utilization, divided into dry and wet methods. Dry baking, also known as mild pyrolysis (200~300℃), is used to remove moisture and improve the quality of solid fuel, with traditional Chinese medicine residue being one of the key research subjects of this technology. Xin et al. proposed that after 240℃ dry baking treatment, the internal cellulose structure of traditional Chinese medicine residue is damaged, leading to the decomposition of a small amount of cellulose, removal of some bound water and light organic matter, an increase in energy density, and the acquisition of high calorific value solid fuel (23.3MJ/kg). This modified fuel can be further rapidly pyrolyzed to produce high-quality bio-oil with fewer oxygen components, indicating that baked medicinal residue has the potential to become an alternative raw material for liquid fuel production. In addition, pretreatment of traditional Chinese medicine residue by dry baking can enhance hydrophobic properties, exhibiting superior combustion behavior (the comprehensive combustion index increases 3-5 times) and gasification performance [optimal gas indicators: yield of 0.86m3/kg, calorific value of 13.7MJ/m3 (standard conditions), tar content of 12.72% (mass fraction)]. Wet baking, also known as hydrothermal carbonization, uses water as a solvent and reaction medium, operating under closed, oxygen-deficient conditions at 150~300℃ and autogenous pressure. In recent years, some research groups have focused on converting high-moisture medicinal residue into modified solid fuel through wet baking, mainly concentrating on quality improvement and nitrogen reduction. Ma et al. showed that under suitable wet baking conditions (180~200℃, 30min), the pre-treatment of bacterial residue results in an increase in the calorific value of the obtained hydrochar and a decrease in nitrogen content; compared to the raw material, burning this hydrochar can reduce NO emissions by 20%-30%. Ahmad et al. reported that both conventional and microwave-assisted wet baking pretreatments of lincomycin bacterial residue can increase the protein nitrogen and pyrrolidone nitrogen content in the coal-water slurry, promoting the conversion of pyrrole nitrogen and pyridine nitrogen to quaternary ammonium salts, ultimately transforming into high-quality hydrochar with a higher fuel ratio and lower nitrogen content. Furthermore, Zhuang et al. and Hu et al. focused on exploring the migration pathways of nitrogen-containing functional groups during the wet baking process of antibiotic bacterial residue, reaching a consistent conclusion: ① Unstable fuel nitrogen is enriched in the form of inorganic nitrogen ions and soluble organic nitrogen in the hydrolysate through hydrolysis or deamination reactions; ② More stable fuel nitrogen or nitrogen-containing intermediates are converted into heterocyclic nitrogen present in the solid-phase hydrochar through condensation and cyclization reactions. These two nitrogen migration pathways reflect the nitrogen reduction potential of wet baking for handling bacterial residue, which is closely related to the reaction temperature. In summary, wet baking modifies medicinal residue by increasing energy density and reducing nitrogen content, making it an effective pretreatment method for disposing of medicinal residue. The latest research further reports a wet baking method involving co-hydrothermal carbonization with lignite, capable of converting medicinal residue into clean, high-quality solid biofuel. The addition of lignite, due to its synergistic enhancement effect on quality improvement and nitrogen reduction, strengthens the relevant hydrothermal reactions, resulting in high-energy-density (24~25MJ/kg) and low-nitrogen-content (mass fraction 1.4%~2.0%) high-quality hydrochar fuel with a stable structure. From these studies, it can be seen that baking, as a thermochemical pretreatment technology, can modify medicinal residue into better solid biofuel by improving the organic component and removing harmful elements. Among them, wet baking seems more suitable for dealing with high-moisture organic solid waste like medicinal residue, as it can avoid the high-energy-consuming pre-drying process. However, this technology also has limitations, as the preparation of enhanced solid fuel often requires more stringent operating conditions (high temperature, long reaction time), inevitably leading to significant yield and energy losses, and also increasing the operational costs of actual baking projects.
In summary, the current research on the utilization of medicinal residue as a solid fuel for energy mainly includes two aspects: ① direct thermal utilization under the principle of low pollutant emissions; ② thermochemical pretreatment, aimed at altering the deficient fuel properties of medicinal residue (such as high moisture content, rich in nitrogen, and abundant in oxygen), focusing primarily on quality improvement and nitrogen reduction. Despite some poor physicochemical properties, the dry state of medicinal residue contains an organic matter mass fraction as high as 90%, with a calorific value reaching 20 MJ/kg, exhibiting excellent biomass characteristics and having potential for energy utilization. Considering the above aspects comprehensively, it can be inferred that using medicinal residue to produce solid biofuel is a feasible method for energy utilization, but not the preferred choice for high-value energy conversion.
03
Preparation process, characteristics, and influence mechanism of gas fuel derived from medicinal residue
3.1
pyrolysis gas production mechanism and product characteristics规律 看起来在翻译时,“规律”这个词没有被转换成英文。让我修正一下: pyrolysis gas production mechanism and product characteristics pattern
Pyrolysis is one of the typical methods for preparing gaseous fuels based on thermochemical conversion. As the most commonly used and cost-effective method, it can selectively convert biomass into non-condensable gases (CO2, CO, CH4, H2, and light hydrocarbons), char, and condensable liquids in an oxygen-free atmosphere. Additionally, it is also the first necessary stage in other thermochemical conversion processes such as gasification or combustion. Previous studies on the preparation of gaseous fuels from medicinal residue via pyrolysis can be summarized into three aspects: the pyrolysis or co-pyrolysis mechanism of medicinal residue, the fuel nitrogen evolution mechanism related to pyrolysis gas phase, and the characteristics of gaseous fuel products from different pyrolysis methods.
Regarding the pyrolysis mechanism of medicinal residue, previous studies have adopted thermal gravimetric (TG) coupling with Fourier transform infrared (FTIR) and/or mass spectrometry (MS) methods, mainly focusing on the kinetic characteristics and thermochemical behavior. For example, Wang et al. studied the pyrolysis mechanism of penicillin mycelial dregs and found that the pyrolysis process could be divided into four stages: water evaporation/light volatile decomposition, main volatile release, carbonization, and inorganic salt decomposition. These stages respectively conform to three-dimensional diffusion, second-order reaction, nucleation-growth, and 1.9th order chemical reaction mechanisms, with corresponding activation energies of 86.01 kJ/mol, 167.87 kJ/mol, 201.79 kJ/mol, and 309.96 kJ/mol. Du et al. explored the characteristics of pyrolysis products from antibiotic mycelial dregs using online methods, finding that when the pyrolysis temperature is below 600°C, nitrogen-containing compounds in the products are mainly NH3, HCN, and HNCO; whereas above 600°C, the main products are H2, CO, and CO2. Additionally, Lin et al. investigated the co-pyrolysis mechanism of traditional Chinese medicine residue and lignite, confirming a synergistic effect between the two: at low-temperature regions, it showed a promoting effect, while at high-temperature regions, an inhibiting effect was observed. It was also found that a 30% to 50% blend ratio of medicinal residue could achieve optimal co-pyrolysis performance, exhibiting a significant kinetic compensation effect.
Summarizing previous relevant research, the pyrolysis mechanism of medicinal residue can be summarized as follows: ① The pyrolysis process generally includes three stages: moisture evaporation, volatilization (main weight loss), and carbonization; ② Reaction kinetics methods are divided into model-free (FWO, KAS) and model-fitting (integral master plots, distributed activation energy) categories, thus the obtained activation energy and frequency factor have a certain range of fluctuation. The reaction order can usually be described by chemical reactions and multi-dimensional diffusion models; ③ Pyrolysis products specifically refer to pyrolysis volatiles (gases and oils), which are mainly determined by temperature and the type of pyrolysis (such as catalytic or co-pyrolysis). Typically, when analyzing pyrolysis oil and gas using MS and FTIR online, a high proportion of nitrogen-containing compounds can be detected. These nitrogen-containing compounds originate from the nitrogen components in the medicinal residue raw material. It is well known that when pyrolysis gas is used as fuel, these nitrogen-containing compounds will eventually convert into NOx pollutants.
Therefore, many studies have been conducted by predecessors on the evolution pathways and patterns of fuel nitrogen related to the pyrolysis process of medicinal residue and nitrogen-containing gaseous pollutants (i.e., NOx precursors), as mainly illustrated in Figure 3.
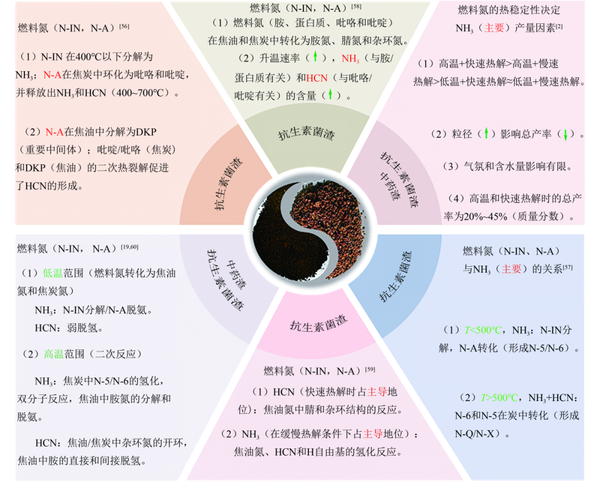
Figure 3
Research perspectives on the evolution of fuel nitrogen related to NOx precursors during the pyrolysis process of medicinal residue
N-IN—Inorganic Nitrogen; N-A—Amine Nitrogen/Aamide Nitrogen/Amide Nitrogen; N-5—Pyrrolic Nitrogen; N-6—Pyridinic Nitrogen; N-Q—Graphitic Nitrogen; N-X—Nitro Nitrogen; DKP—2,5-Diketopiperazine
Based on the above research, the following consistent conclusions can be drawn: ① The main types of fuel nitrogen are amine/amide/amino nitrogen and some inorganic nitrogen such as ammonium salts; ② The evolution pathway of fuel nitrogen is during the initial pyrolysis (devolatilization) stage, where fuel nitrogen, based on thermal stability differences, undergoes bond cleavage reactions and cross-linking reactions to convert into tar nitrogen (amine nitrogen, heterocyclic nitrogen, nitrile nitrogen) and char nitrogen (pyrrole nitrogen, pyridine nitrogen). In the secondary reaction stage, the three major components of tar nitrogen further undergo cracking or condensation, while char pyrrole/pyridine nitrogen evolves into larger and more stable heterocyclic nitrogen (graphitic nitrogen and nitride nitrogen); ③ The formation of NOx precursors, HCN is mainly formed in the secondary reaction stage, more dependent on the thermal cracking of large tar nitrogen components, NH3 can be generated in both stages, with primary pyrolysis related to the thermal stability of fuel nitrogen, and secondary reactions depending on the thermal cracking of char nitrogen/tar nitrogen components; ④ The main influencing factors include thermal conditions such as temperature, type of pyrolysis, and heating rate. Accelerating the heating rate or increasing the temperature will promote the generation of NOx precursors.
From the evolution path and pattern of fuel nitrogen, it can be seen that the source-end regulation of NOx precursors in the pyrolysis process should achieve a reduction in nitrogen pollutants from the thermal utilization of medicinal residue. Zhan et al. explored two types of pyrolysis technologies for regulating NOx precursors in antibiotic mycelial residue: on one hand, using low-temperature pyrolysis (250~300℃), effective removal (31%) of fuel nitrogen in medicinal residue can be achieved with a smaller energy loss (25%); on the other hand, by employing staged pyrolysis to regulate the intensity of reactions related to the formation of NOx precursors at different stages, their yield can be reduced by about 36%. The above-mentioned regulation methods further analyze the relationship between the intensity of pathway reactions and the yield of NOx precursors from the perspective of pyrolysis methods, but they do not consider much the regulation of the generation or conversion of NOx precursors with the goal of harmless transformation of fuel nitrogen. Future research should focus on the principle of harmless transformation of fuel nitrogen during the pyrolysis of medicinal residue, and carry out effective regulation of the generation and conversion of NOx precursors.
Regarding the study of gas fuel properties, many scholars have explored the possibility of enhancing their quality through different pyrolysis methods, such as catalytic, microwave, co-pyrolysis, and their coupling methods, with the main raw material being traditional Chinese medicine residue. Zhang et al. found that microwave-induced catalytic (ZSM-5) pyrolysis under a CO2 atmosphere can produce high-quality pyrolysis gas, with CO and H2 accounting for about 20.1%. Ding et al. investigated in-situ CO2 adsorption pyrolysis of traditional Chinese medicine residue with CaO participation, proving that CaO promotes the water-gas shift reaction by adsorbing CO2, methanation, and large molecule conversion, significantly improving the quality of catalytically cracked gas. Zhao et al.'s research reached similar quantitative conclusions; under optimal conditions (700°C, Ca/H=0.65), high-value pyrolysis oil could be obtained, with a calorific value of 18.1 MJ/m³ (standard conditions), and combustible components exceeding 90%. Xu et al. further used Ni/CaO catalysts for catalytic pyrolysis of traditional Chinese medicine residue, finding that adding the active component Ni improves CaO's resistance to deactivation in cyclic CO2 absorption. At 700°C, adding 10% (mass fraction) of Ni component effectively removes CO2 from the pyrolysis gas, obtaining high-calorific (18.6 MJ/m³) and high-yield (325.3 mL/g) gas fuel. Li et al. chose a co-pyrolysis strategy of high-ash papermaking sludge with traditional Chinese medicine residue, discovering that adding 50% papermaking sludge promotes the generation of H2 and CO2, producing more gaseous products, improving the deoxygenation and aromatization of heteroatom tar, and reducing tar production. Li et al. selected Ni-based catalysts for catalytic pyrolysis of traditional Chinese medicine residue assisted by microwaves, showing that the combined effect of both can reduce coke yield and NOx emissions by approximately 74% and 37%, respectively, while increasing gas yield by about 143%. Additionally, Zheng et al. studied the co-pyrolysis of antibiotic mycelial dregs and wood chips, finding that the synergistic effects during the process not only promoted the generation of gaseous products but also reduced coke production. Therefore, future research can focus on non-traditional pyrolysis technologies, aiming to enhance the production of combustibles (CO, H2, CH4) by reducing useless and harmful components (CO2, tar, nitrogen-containing substances), thereby achieving an improvement in the quality of pyrolysis gas.
It has been proven that pyrolysis, based on an anaerobic environment and driven by thermal and self-pyrolysis mechanisms, is a technology capable of efficiently converting medicinal residue into high-quality gaseous fuels. Previous studies on the kinetics of pyrolysis and thermal degradation behavior help clarify the formation pathways and influencing factors of pyrolysis gases, thereby facilitating the selection of appropriate pyrolysis technologies for different types of medicinal residues. In the future, attention should be paid to controlling the generation and effective conversion of undesirable components in pyrolysis gases (such as CO2, NOx precursors, and tar-like substances) while ensuring the yield of gaseous fuels.
3.2
Characteristics and influencing factors mechanism of gasification process for medicinal residue
Biomass gasification is a thermochemical conversion technology that transforms raw materials into combustible gas under high-temperature and oxygen-deficient conditions. For the energy utilization of medicinal residue, gasification technology shows application potential in the following aspects: ① short reaction time, enabling large-scale industrial production; ② high reaction temperature, allowing for the decomposition and destruction of organic pollutants such as antibiotics; ③ flexible and simple operation, conducive to energy recovery; ④ strong adaptability to high-moisture biomass waste.
Previous research on the gasification of medicinal residue has primarily focused on traditional Chinese medicine residue, evolving from laboratory studies to engineering demonstrations, with the aim of increasing the energy density of combustible gases and reducing tar content. Zeng et al. demonstrated the feasibility of a two-stage fluidized bed system for gasification, obtaining optimal operating conditions through laboratory studies: pyrolysis temperature 700°C, gasification temperature 850°C, equivalence ratio 0.04, and residence time 0.9s. Under these operating conditions, a certain autothermal demonstration system can achieve a processing capacity of 0.6t/h, with the calorific value of the produced gas and tar content being 5.0MJ/m3 (standard conditions) and 0.4g/m3 (standard conditions), respectively. Dong Yuping's team systematically explored the impact of relevant factors in a pilot-scale circulating fluidized bed gasification system, including air equivalence ratio, steam-to-biomass ratio (S/B), and feedstock properties. The experimental results showed that under conditions of low moisture content in the feedstock (<9%), small particle size (<4mm), appropriate equivalence ratio (0.24~0.40), and suitable S/B (0.23~0.43), the system could reach optimal gasification performance, with a processing capacity of 210~352kg/h. In another engineering study, they further confirmed these findings, with the gasification system achieving a processing capacity of 300kg/h and continuous operation for 200 hours. At an equivalence ratio of 0.35 and an average feed rate of 5.5kg/(m2·s), the circulating fluidized bed achieved the best gasification performance, with the calorific value of the produced gas at 4~5MJ/m3 (standard conditions) and a cold gas efficiency of 62.36%. Additionally, it was found that a certain level of moisture and ash components (metal oxides) in the medicinal residue, due to their catalytic effect, could promote effective tar decomposition, thereby improving the quality of the produced gas. Wang et al. studied the gasification experiment of Tibetan plateau traditional Chinese medicine residue (under local pressure) and found that at an equivalence ratio of 0.24 and a gasification temperature of 1000°C, the calorific value of the produced gas could reach 5.59MJ/m3 (standard conditions), with a cold gas efficiency of 63.22% and a carbon conversion rate of 68.87%. Guo et al.'s research indicated that under the influence of different gasifying agents, the air equivalence ratio could be optimized to 0.26, with the order of influence of gasification parameters being S/B > O2 concentration > temperature > air equivalence ratio. Wang Yin et al. proposed a two-step gasification system coupling a fluidized bed and a fixed bed, finding that extending the residence time or introducing a certain amount of steam/oxygen is beneficial for tar cracking and char gasification in the fixed bed. Guan et al. investigated the impact of dual circulating fluidized bed operating parameters on the gasification characteristics of traditional Chinese medicine residue, discovering that under conditions of temperatures between 750~800°C, feedstock moisture content by mass fraction of 5%~10%, an equivalence ratio of 0.23~0.26, and S/B of 0.4~0.6, the optimal gasification effect could be achieved (calorific value of the produced gas >8MJ/m3, energy efficiency >75%).
Recently, Dong et al. proposed an integrated energy conversion system for the utilization of traditional Chinese medicine residue (Figure 4), which has achieved good results on a small experimental setup: ① Gasification feedstock, with particle size less than 10mm and moisture content of about 20% (mass fraction); ② Gasification characteristics, with a gasification efficiency of 76.1%, calorific value of the produced gas at 5.7MJ/m³, and gas yield of 0.6m³/kg (under standard conditions) of feedstock; ③ Integrated energy effect, for every 1kg of feedstock processed, 0.3292kg of steam can be produced, with a net energy output of 0.89MJ and 1.0167kg of wastewater.
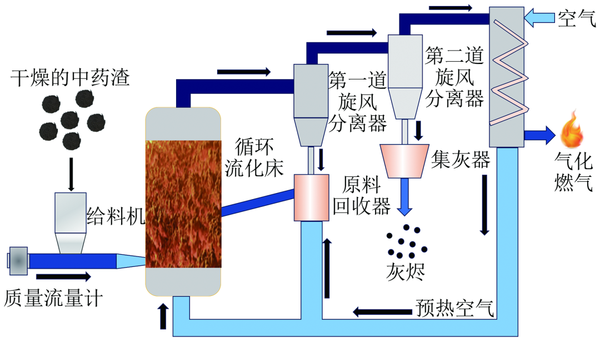
Figure 4
integrated energy conversion system for gasification and heat supply of traditional Chinese medicine residue
In addition, regarding the gasification application of antibiotic bacterial residue, because it belongs to non-woody cellulose and is rich in fuel nitrogen, only a few studies have been involved. Du et al. believe that when adding antibiotic bacterial residue to an industrial-scale water-coal slurry gasifier for co-gasification, it will not change the composition of the gasification gas, and pollutant emissions will not exceed standards. Obviously, this study did not consider the impact of NOx precursors from fuel nitrogen during the co-gasification process when evaluating gaseous pollutants. Liu et al. studied the chemical looping gasification of bacterial residue and red mud, finding that with an oxygen carrier/fuel ratio of 4:1 and the addition of a certain amount of steam, the gas yield and H2 concentration can be maximized. However, due to the enhanced effect of the oxygen carrier on the conversion of fuel nitrogen, the concentration of NOx pollutants in the gas is very high.
In summary, the existing research on the gasification of medicinal residue mainly focuses on two objectives: improving the quality of the gas and reducing the tar content. In terms of fundamental research, the influence of factors such as temperature, gasifying agent, equivalence ratio, S/B, moisture content of the raw material, and particle size on these two objectives has been explored. From an engineering application perspective, the feasibility and stability of gasifying medicinal residue using different scale demonstration systems based on single and dual-loop circulating fluidized beds, dual fluidized beds, and two-stage beds have been demonstrated. From these studies, the following measures can be taken to reduce tar content and improve gas quality: ① Design the system so that related gasification reactions (raw material pyrolysis, char gasification, volatiles reforming) do not occur in the same area or at the same time; ② Utilize the surface catalytic and redox effects brought about by the recirculation of components (such as ash) in conjunction with steam. Therefore, future gasification technologies based on the concepts of reaction decoupling and process intensification should enable efficient energy utilization of medicinal residues. However, it is worth noting that for nitrogen-rich medicinal residues, the implementation of the above gasification concepts requires priority consideration of the harmless disposal of fuel nitrogen.
04
Preparation methods and influencing factors of liquid fuel derived from medicinal residue
Biomass-based liquid fuels mainly refer to bio-oil, a dark brown liquid with a distinctive smoky odor, composed of hundreds of organic compounds (such as acids, oxygenates, phenols, sugars, and high molecular weight oligomers), typically produced through the fast pyrolysis or hydrothermal liquefaction of biomass. Bio-oil generally has two main uses: ① direct combustion as boiler fuel; ② upgrading and refining to produce high-quality diesel for vehicles. So far, some basic research work has been carried out by scholars on the conversion of medicinal residue into bio-oil, mainly focusing on the characteristics and upgrading technologies of bio-oil prepared from two types of medicinal residues.
For traditional Chinese medicine residue, pyrolysis temperature is a key factor in determining the composition and yield of bio-oil. In addition, pretreatment methods such as steaming, extraction, and roasting also affect the quality of the bio-oil. Wang et al. found that the effect of steaming treatment on medicinal residue is similar to that of temperature rise, which can increase ketone production and inhibit the formation of sugars and furans. Zhang et al. used ethanol for the extraction pretreatment of medicinal residue, increasing the yields of ketones, acids, and furans in low-temperature pyrolysis bio-oil by 11%, 14%, and 41%, respectively. Xin et al. found that the main components of bio-oil obtained under medium-temperature conditions (400~600°C) are acids, ketones, and anhydrous sugars, while bio-oil obtained from high-temperature pyrolysis mainly consists of phenols, aromatics, and nitrogen-containing compounds, further demonstrating that high-temperature pyrolysis is more conducive to the formation of larger aromatic ring structures. Moreover, catalytic fast pyrolysis technology can be used for the upgrading and refining of bio-oil. Zhang et al. found that using ZSM-5 catalyst under CO atmosphere can obtain more stable bio-oil with higher hydrocarbon ratios and lower oxygenated compounds; Lee et al. used palladium carbon catalysts, which effectively inhibited the formation of benzene substances in bio-oil, reducing harm to the environment and human health. Li et al. significantly increased the content of aromatic hydrocarbons in bio-oil using Ni-Fe/CaO-Al2O3 catalyst in an in-situ reaction system, thereby improving the quality of bio-oil. The use of nickel-aluminum oxide (20%)/MCM-41 composite material can increase the yield of bio-oil by about 15% (compared to non-catalytic processes), with the content of aromatic compounds exceeding 90%. This shows that the effect of catalyst supports on the upgrading and refining of bio-oil is significant, in the order of Al2O3>Al-SBA-15>ZSM-5. The best-supported catalyst can increase the yield of bio-oil by 34.26%, raise its calorific value to 25.94 MJ/kg, and reduce oxygen content to as low as 26.71% (by mass). Research groups have successfully applied catalytic pyrolysis systems to the clean production of bio-oil, capable of processing 1.14 million tons of traditional Chinese medicine residue annually, saving 0.914622 million tons of standard coal, and reducing CO2 emissions by 1.72 million tons. Additionally, co-pyrolysis and catalytic hydrothermal liquefaction have also been proven feasible. Co-pyrolysis of low-density polyethylene with traditional Chinese medicine residue can increase the content of aliphatic hydrocarbons and alcohols in bio-oil. Guan et al. used Fe/ZSM-5 catalyst for the catalytic hydrothermal liquefaction of traditional Chinese medicine residue, achieving a high heating value of 31.74 MJ/kg for the bio-oil, with aliphatic compounds accounting for 72.96%.
Some teams have studied the production of bio-oil from antibiotic mycelial dregs using fast pyrolysis and hydrothermal liquefaction technologies. The resulting bio-oil is mainly composed of nitrogen-containing compounds derived from fuel nitrogen, including amides, amines, pyridines, and indoles. When the pyrolysis temperature is 400°C, the highest yield of bio-oil reaches up to 57.3%; when the temperature is 600°C, the nitrogen content is as high as 8.9% (mass fraction). Meanwhile, through quantum chemistry and classical variational theory analysis, it has been proven that the nitrogen-containing compounds originate from the specific migration and transformation of proteins and their hydrolysis products in the raw materials. In addition, to improve the quality of bio-oil, some scholars have further explored the optimal operating conditions for hydrothermal liquefaction in the production of bio-oil. Under optimized temperature, residence time, and total solid content, the yield of bio-oil can reach 25%~35%, with a calorific value exceeding 30 MJ/kg. Besides abundant oxygenated aliphatic/aromatic hydrocarbons, the bio-oil also contains a considerable amount of nitrogen-containing compounds.
Currently, the value-added strategy of converting medicinal residue into bio-oil through fast pyrolysis and hydrothermal liquefaction technology faces opportunities and challenges: ① The upgrading and refining of bio-oil must start from the perspective of deoxygenation and denitrogenation, developing more efficient catalytic strategies to achieve value-added applications of liquid fuels; ② A large number of nitrogen-containing heterocycles in bio-oil can be produced through a nitrogen value-added strategy using medicinal residue (especially antibiotic mycelial residue) to produce high-value nitrogen-containing compounds for the pharmaceutical, agrochemical, and polymer industries, which is worthy of attention and promotion.
05
Comparison of Thermochemical Conversion Preparation Strategies for Derivative Modified Fuels from Medicinal Residue
In summary, the research shows that through dry and wet thermochemical conversion (roasting, pyrolysis, liquefaction, gasification) strategies, the preparation of solid, gaseous, and liquid derived fuels from medicinal residue can to varying degrees achieve the resource utilization of such waste, with the following advantages: on the one hand, technologies such as pyrolysis and gasification can directly degrade residual antibiotics, antibiotic resistance genes, mycotoxins, and other pollutants in the medicinal residue, effectively addressing the environmental issues caused by the "waste" attributes of medicinal residue; on the other hand, technologies such as dry and wet roasting and hydrothermal liquefaction can overcome the typical defects of medicinal residue, such as high moisture content and low energy density, allowing for the acquisition of modified high-value fuel without the need for additional energy consumption for drying. Figure 5 summarizes the advantages and disadvantages of the three types of derived modified fuel thermochemical conversion technologies.
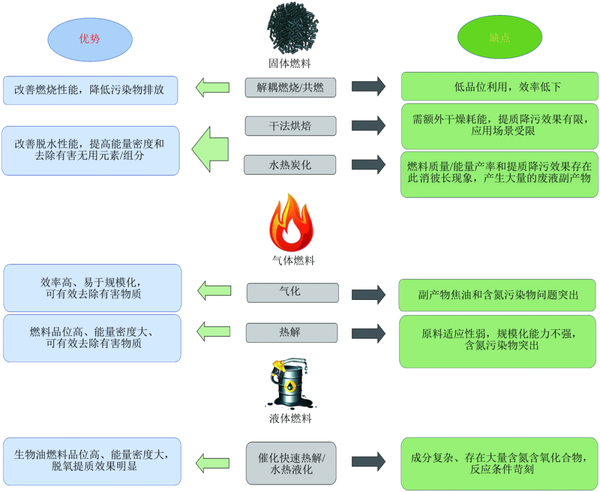
Figure 5
Comparison of thermochemical conversion preparation strategies for modified fuels derived from medicinal residue
As shown in Figure 5, the relevant thermochemical conversion technologies each have their advantages and disadvantages for realizing the resource utilization of medicinal residue based on derived modified fuels. From the perspective of thermochemical process parameters and product characteristics, there are common issues with using a single thermochemical conversion strategy to handle medicinal residue: ① Due to the high nitrogen content in the medicinal residue, various fuel products generally contain a large amount of nitrogen components, which poses a challenge for ultra-low emission of nitrogen pollutants at the backend; ② The majority of single thermochemical strategies for preparing derived fuels produce a large amount of by-products or waste liquids, making it inevitable to seek effective secondary disposal solutions. Focusing on the waste defect attributes of medicinal residue, if we start from the comprehensive utilization of all components, hydrothermal technology coupled with subsequent thermochemical conversion technology should be able to achieve the goal of clean and efficient resource utilization under the premise of meeting pollutant control requirements. The liquid phase aims at water-soluble, nitrogen-rich liquid fertilizers, while the solid phase is selectively directed towards clean, high-value fuels, carbon materials, or solid carbon fertilizers, achieving co-production. The targeted regulation and efficient conversion of valuable components/elements in the associated processes will be the focus of future research on this strategy.
06
conclusion
Medicine residue has dual attributes of resource and waste: on one hand, due to its richness in organic matter, its waste attribute is superior to conventional organic solid wastes such as sludge, livestock and poultry manure, and household waste; on the other hand, due to characteristics like high water content, high nitrogen, and rich oxygen, its resource attribute is inferior to conventional woody and herbaceous biomass. Focusing on these dual attributes, existing research, based on the principles of "reduction, harmlessness, and resource utilization," has carried out work on the preparation, properties, and applications of solid, liquid, and gaseous fuels derived from medicine residue. The main conclusions are as follows.
Converting medicinal residue into fuel products, especially solid fuels, is a direct and appropriate choice for large-scale resource utilization of medicinal residue at the current technological level, with both technical and economic feasibility. However, the aforementioned defect attributes of medicinal residue are the main obstacles to its conversion into target fuels and achieving clean and efficient energy utilization. For solid fuels, some pretreatment methods such as dehydration and torrefaction can enhance product performance by improving quality and reducing nitrogen, but the effects are limited and bring about the problem of secondary byproduct disposal; pyrolysis, gasification, or hydrothermal liquefaction can effectively convert medicinal residue into gaseous and liquid fuels with higher energy density, but the migration, transformation, and presence of harmful or useless components (especially nitrogen) during the process will adversely affect the quality or yield of the resulting fuels (pyrolysis gas, syngas, and bio-oil) to varying degrees. Therefore, to achieve efficient and emission-compliant energy utilization of medicinal residue, future research should focus on addressing these defect attributes, exploring more economical and environmentally friendly thermochemical strategies to realize the conversion of medicinal residue into high-value derived fuels. Additionally, considering the regular mechanisms of hydrothermal technology in dealing with defect attributes, if hydrothermal processes are coupled with other thermochemical conversion methods, aiming for the comprehensive utilization of medicinal residue and synergistic pollution control, co-producing high-value fuels and non-fuel products (carbon materials, solid-liquid fertilizers), it should ensure the efficient conversion of valuable components of medicinal residue while guaranteeing the targeted regulation of harmful/useless components, which represents a potential direction for future research.
【Copyright and Disclaimer】The above information is collected and organized by PlastMatch. The copyright belongs to the original author. This article is reprinted for the purpose of providing more information, and it does not imply that PlastMatch endorses the views expressed in the article or guarantees its accuracy. If there are any errors in the source attribution or if your legitimate rights have been infringed, please contact us, and we will promptly correct or remove the content. If other media, websites, or individuals use the aforementioned content, they must clearly indicate the original source and origin of the work and assume legal responsibility on their own.
Most Popular
-
Covestro faces force majeure!
-
Breaking News! Mitsui Chemicals TDI Unit in Japan Experiences Chlorine Gas Leak Accident!
-
Mitsubishi Chemical Exits! Sumitomo Acquires!
-
DuPont plans to sell Nomex and Kevlar brands for $2 billion! Covestro Declares Force Majeure on TDI / oTDA-based / Polyether Polyol; GAC Group Enters UK Market
-
Borealis suspends polyolefin recycling plant in Austria, Hyundai achieves record Q2 revenue, Volkswagen lowers performance expectations